The year 2021 will see the 50th anniversary of Nixon’s declaration of the war on cancer. The celebration is unlikely to make for a fun time. It was not officially called a war, but a massive effort was undertaken, and while we’ve conquered new scientific territories — exploring the opportunities and risk of genetic engineering — it is clear that a victory celebration is not in view. My memory of the time was that scientists warned it was premature to look for a “cure.” In the end, we invested much in basic science, the suggested alternative, but we are still pretty much in the dark regarding a comprehensive understanding.
A common description of the problem is that we put too much emphasis on the so-called somatic mutation theory, the idea that single or multiple mutations in particular cells lead to uncontrolled proliferation, resistance to inhibition, and the other “hallmarks of cancer,” as described by Douglas Hanahan and Robert Weinberg (1). There is a realization that, intentionally or otherwise, the importance of a metabolic approach had been downplayed. It is not always easy to distinguish between the genetic and metabolic effects — if we think of DNA as the blueprint, the cellular factory sends the product (proteins and other metabolites) back to the blueprint for new instructions all the time. In any case, the 2011 update on hallmarks (2) emphasized disruption in metabolic pathways as one of the key features of the cancerous state. Emphasizing metabolism represents a top-down approach and is more likely to provide a key to nutritional approaches to cancer treatment.
The change in focus was precipitated by the appearance of the ghost of Otto Warburg. Around 1920, Warburg noticed cancer cells have a different energy metabolism than normal cells — they seemed to be able to rely on anaerobic metabolism (without need for oxygen) even if oxygen was present. He thought this was the characteristic feature of all cancer cells. That turned out to be an exaggeration, but the nature of the Warburg effect and the metabolic mechanism that might cause it is on the minds of cancer researchers. What could account for this change in metabolism? What step is going wrong?
The focus on energy metabolism got a lot of us thinking about ketogenic diets. The most obvious function of such a diet is to switch your body to a different fuel. With regard to cancer, the promise is that ketone bodies represent a fuel that the host will prefer (human biology had millions of years to work it out) while the cancer cell will not have experienced any selective pressure in the modern world to adapt to the ketotic state and might even be inhibited.
Energy Metabolism: The Black Box of Life
Regarding energy metabolism, we all know the basic idea: For humans, food and oxygen are taken in, water and carbon dioxide are expelled, and somehow the transformation provides energy for life processes. Graphically, consider the “black box of life,” and for simplicity, focus on glucose. In a black-box or “systems” approach, we look at inputs and outputs and see what we can learn under conditions where we don’t have enough information about mechanism.
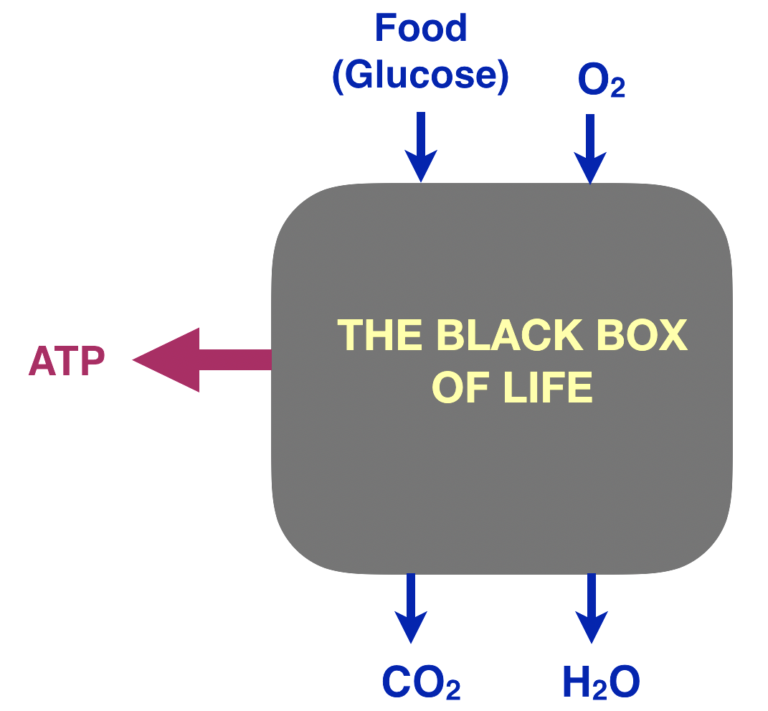
Figure 1: The black box of life
The process is oxidation — specifically, the combination of atoms of glucose with molecular oxygen (O2).
glucose + O2 ➛ CO2 + H2O
The reaction is highly exergonic (i.e., it generates energy). Roughly speaking, we identify energy in biologic systems with the presence of the compound ATP (adenosine triphosphate).
The energy from metabolism is coupled to the phosphate transfer reaction ADP + Pi ➛ ATP + H2O, where ADP stands for adenosine diphosphate and Pi for inorganic phosphate. Simplified bottom line: the extent of the ATP-to-ADP ratio gives us a rough idea of the energy state of the cell.
The question is how oxidation of glucose leads to phosphorylation of ATP.
Energy Metabolism Has Two Big Stages
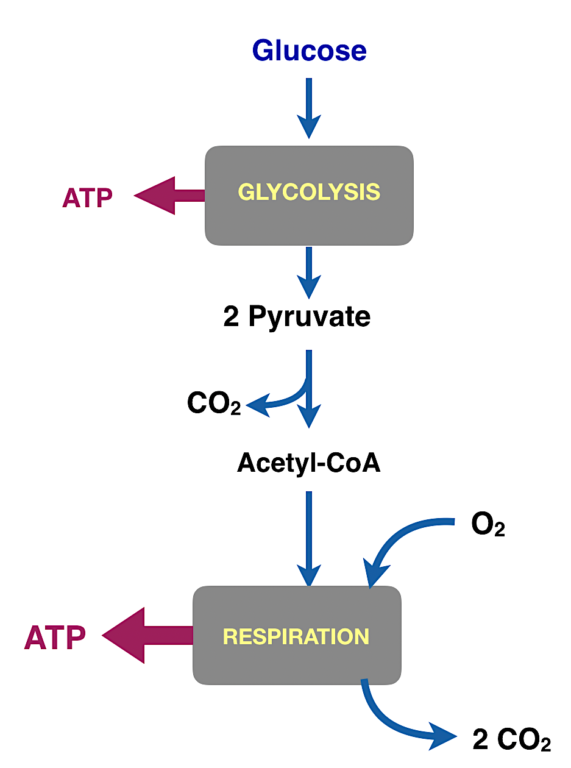
Figure 2: The major processes in energy metabolism. Glycolysis (glyco-, sugar; lysis, cutting) converts the six-carbon compound glucose into two molecules of the three-carbon compound pyruvate. Pyruvate is converted to a derivative of acetic acid called acetyl-CoA.
For living organisms, getting the energy out of an oxidation reaction in a useful form is tough. You can’t run things at high temperatures as we do in the combustion engines we make ourselves. And storing the energy in a small molecule like ATP is difficult to even think about. Of course, evolution had six million years to work it out, but it is still a remarkable thing. It has to be done in small steps.
The energy is collected into metabolic pathways. The two major pathways for energy metabolism are glycolysis and respiration.
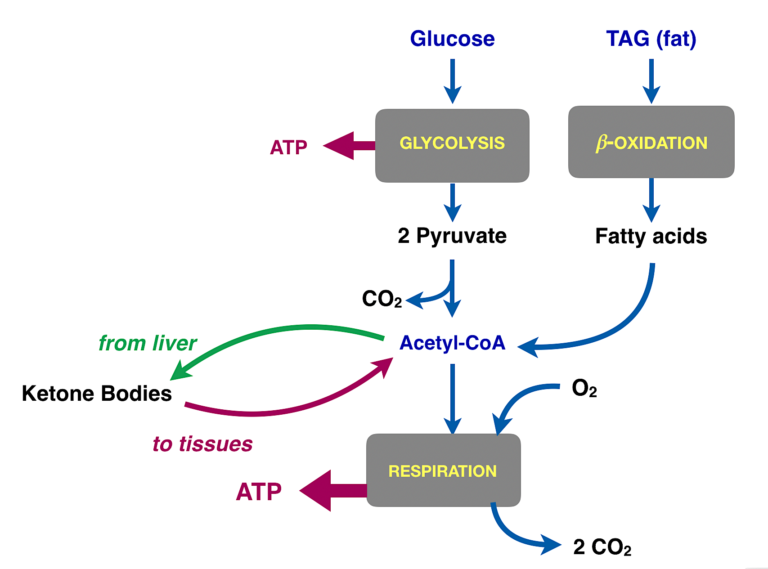
Figure 3: Summary of different fuels in metabolism. The main goal of glycolysis in most cells is to prepare the carbons of glucose for oxidation in respiration. The actual substrate for respiration is acetyl-CoA, which is the product of glycolysis. Acetyl-CoA is also provided by fatty acids from fat. Acetyl-CoA in the liver can be assembled into ketone bodies. The green arrow represents export of ketone bodies from the liver. The red arrow is meant to represent the transport of the ketone bodies into other tissues, especially muscle and brain. In these tissues, the ketone bodies are converted back to acetyl-CoA and into respiration (The liver doesn’t use ketone bodies because it already has acetyl-CoA).
Why Do We Think Ketogenic Diets Will Be Useful for Treating Cancer?
The Warburg effect is often described as the reliance of cancer cells on so-called “aerobic glycolysis” — i.e., glycolysis that occurs even in the presence of oxygen. Glycolysis is anaerobic but if oxygen is available, normal cells will use the pyruvate, the product of glycolysis for respiration. A general description of the Warburg effect is that somehow, the connection between glycolysis and respiration became corrupted. The obvious place to look is at the connection point, the step where we convert pyruvate to acetyl-CoA. If the conversion is incomplete, other products, in particular lactate, will be generated from the pyruvate. That’s what Warburg actually found. He measured the CO2 released from cancer cells in culture as an indicator of respiration, and he compared that to the lactate produced. The ratio of CO2 to lactate was higher in cancer cells than normal cells.
So acetyl-CoA is oxidized to CO2, and that provides most of our energy (ATP). We can get acetyl-CoA from glycolysis (via pyruvate), but there are other sources. In particular, fat or ketone bodies (Figure 3) are major sources. The liver makes acetyl-CoA from incoming fat. The acetyl-CoA provides fuel for the liver itself but under conditions of starvation or low carbohydrate intake, liver enzymes convert some of the acetyl-CoA to ketone bodies for export. The big take-home message: The function of ketone bodies is to transport acetyl-CoA from the liver to the tissues.
Now, we think that if we reduce the total amount of carbohydrates coming in (directly, or by reducing total calories) we can switch to using acetyl-CoA from fat or ketone bodies, and thus we can bypass whatever went wrong in the development of cancer. We know that the host has evolved over centuries to use ketone bodies, whereas we think of the cancer as a new species, evolving, in a way, within the lifetime of the individual. On this basis, we expect that the host will have the advantage if ketone bodies become a major fuel and thus can compete with the tumor. In addition, we have proven by experiment that a switch in metabolism from higher carbohydrate to lower carbohydrate or to a ketogenic state can have dramatic effects in diabetes and many cases of epilepsy, and such a switch is implicated in numerous other conditions. We anticipate unexplored metabolic outcomes as cells adapt to the ketogenic environment. Of course, they could be the wrong outcomes, but we think evolution is on our side. Ketosis was a way of life for our prehistoric ancestors.
Finally, if we can put a tumor at a competitive disadvantage in sources of energy, it may become vulnerable to other modalities of inhibition, chemical or otherwise (In vivo, tumors cannot be starved for glucose because glucose is always available in the blood, but we can provide more efficient fuels for the host).
Calories, Carbohydrate, and Ketone Bodies
Total caloric restriction is the only universally acknowledged lifestyle intervention that can be beneficial for resisting or curing cancer. Whether the de facto reduction in carbohydrate that must accompany such calorie restriction has a causal role is not usually addressed (If the baseline is a high-carbohydrate diet and you reduce calories across the board, the major reduction will be in carbohydrate). A direct example, however, goes back to experiments done by Albert Tannenbaum in 1945 (3). He grafted skin tumors onto mice who had been kept on control diets or those with reduced calories or specific carbohydrate restriction (Figure 4). He found, “The mice receiving the diet restricted in carbohydrate only developed fewer tumors, and at a later time of appearance, than did the mice receiving the diet restricted in all components” (3, p. 617).
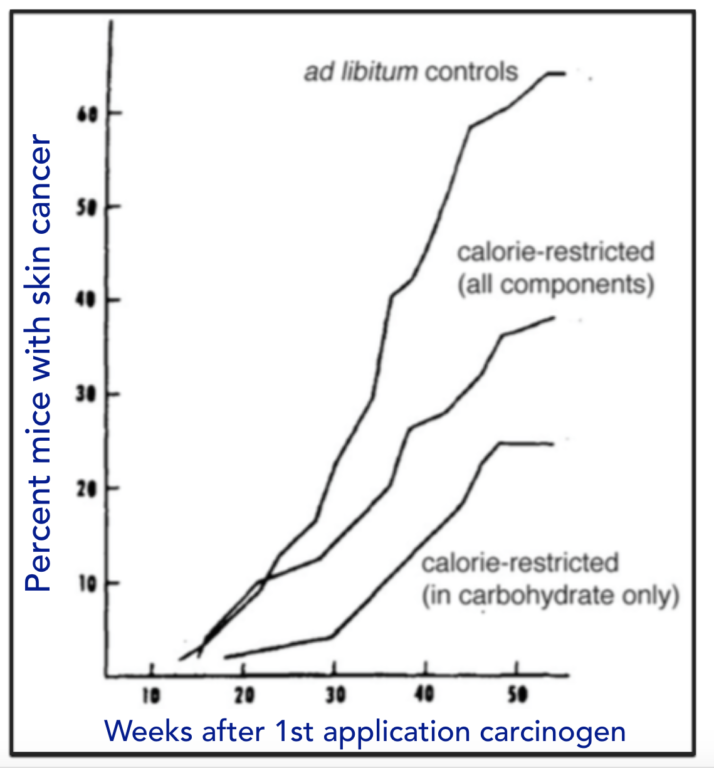
Figure 4: Effect of diet on induced skin cancer. Mice were kept on indicated diets for two weeks and then treated with a carcinogen (benzypyrene) and presence of tumors examined.
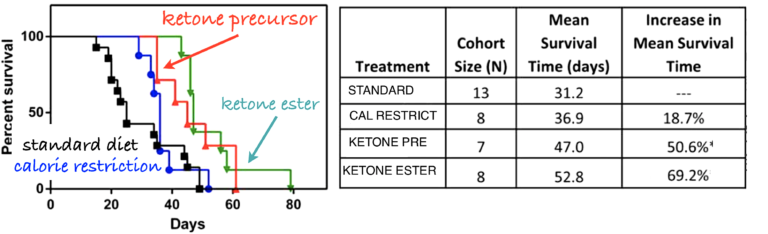
Figure 5: Adult male inbred mice were implanted with cancer cells from similar mice. The mice were then put on one of four diets: standard diets, calorie-restricted diets, or diets supplemented with compounds that gave rise to ketone bodies.
Similar results from several groups support the potential of carbohydrate restriction. In the case where the low-carbohydrate diet actually leads to ketosis, there is always the question as to whether any effects are due to the removal of carbohydrate or to the direct effect of the ketone bodies. Angela Poff and Dom D’Agostino fed mice diets supplemented with compounds that produced ketone bodies (4). They found extended survival in the supplement-receiving mice, compared to controls on a standard diet (Figure 5).
Other Fates of Glycolysis?
Not all organisms, and in fact not all human tissues, require oxygen to obtain ATP. All living cells carry out glycolysis, but some cells do not put the pyruvate into aerobic metabolism. In microorganisms, glycolysis is the process underlying fermentation. Fermenting yeast and bacteria convert pyruvate to alcohol. Lactic acid bacteria, in yogurt for example, convert pyruvate to lactic acid. Rapidly exercising muscle or red blood cells can metabolize glucose without a requirement for oxygen and produce lactic acid as the byproduct. Glycolysis provides a certain amount of ATP, and the reaction is, in fact, an oxidation reaction. So, how do you oxidize something without oxygen? To understand this, we need to take a closer look at oxidation.
Oxidation: The Next Level
Carbohydrates and fats, which make up the majority of our dietary fuel, are mostly carbon and hydrogen. The black box of life shows that the carbons are oxidized to CO2, but if something has been oxidized, something else must have provided the oxygen and that thing, the oxidizing agent, must have been reduced. In the case of the black box of life (food + oxygenic in; water and CO2 out), some of the oxygen atoms themselves are reduced to water.
The paragraph above presents the definition of reduction: giving up oxygen or adding hydrogen. So, oxidations are always part of oxidation-reduction reactions, or redox for short, even if we focus on one or another part of the reaction. Redox reactions are a fundamental part of chemistry, but in biochemistry and metabolism, we have these chemical definitions:
Oxidation = combination with oxygen (O) or loss of hydrogen (H)
Reduction = combination with H or loss of O
The oxidation of glucose proceeds by two major processes. As described above, glycolysis does not require oxygen and converts the six-carbon compound glucose to two molecules of the three-carbon compound pyruvate (pyruvic acid). Using the rule that increased combination with oxygen or fewer bonds to hydrogen constitutes oxidation, pyruvate is overall oxidized compared to glucose. There must be a different oxidizing agent than molecular oxygen. It turns out metabolism has evolved several; the most common is NAD. A somewhat complicated molecule, the good news is that it is always written as the abbreviation and exists in an oxidized form, written NAD+, and in a reduced form, NADH. So, the real reaction for glycolysis is as follows:
Glucose + NAD+ ➛ pyruvate + NADH
Now, under normal oxidative metabolism, the NADH gets re-oxidized. In other words, despite the straightforward appearance of the black box, the actual mechanism inside is indirect. The oxygen never sees the food. There is an intermediate, a redox coenzyme, that does the (relatively low-energy) oxidation and is itself the target for oxidation by oxygen. The picture is an agent, NAD shuttling back and forth between food (which gets oxidized) and oxygen (which puts the NAD back in the oxidized form).
Where Does Lactic Acid Come From?
What happens in a cell that does not have the biochemical machinery to convert pyruvate to acetyl-CoA and does not have the machinery to re-oxidize the NADH back to the oxidizing agent NAD+? What does an anaerobic cell do with the NADH from glycolysis? In the case of microorganisms, the NADH is used to convert pyruvate to one or another end product, the most familiar being alcohol in fermenting organisms. Some bacteria and some human cells use the NADH to reduce pyruvate to lactate. This is what Warburg saw. His cancer cells were overproducing lactate and underproducing carbon dioxide.
What Went Wrong in Cancer and Can Ketone Bodies Help? Looking Ahead
Why does the cell switch to lactate in the cancerous state? What is the advantage for such a cell? What is the role of the mitochondrion? How could ketone bodies help? Such questions will be explored in the next module.
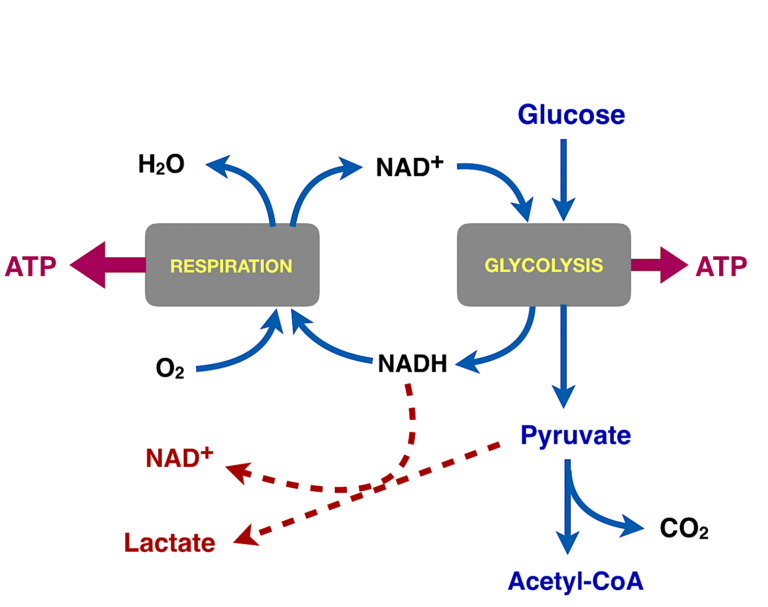
Figure 6: Summary of glycolysis and fates of pyruvate. Glucose is oxidized in glycolysis to pyruvate. In normal aerobic cells, pyruvate is converted to acetyl-CoA and oxidized to carbon dioxide (also by NAD+, not shown), and the NADH from glycolysis (and from the aerobic conversion to carbon dioxide) is oxidized by molecular oxygen. In cancer, in the Warburg effect, this process is disrupted somehow and pyruvate uses the NADH to produce lactate.
References
- Tannenbaum A. The dependence of tumor formation on the composition of the calorie-restricted diet as well as on the degree of restriction. Amer. Assoc. for Cancer Research 5(1945): 609-615. Available here.
- Poff A, D’Agostino D, Ari C, Arnold P, Seyfried TP. Ketone supplementation decreases tumor cell viability and prolongs survival of mice with metastatic cancer. Int. J. of Cancer 135.7(14 May 2014): 1711-1720. Available here.
Richard David Feinman, Ph.D., is a professor of cell biology at the State University of New York Downstate Medical Center in Brooklyn, where he has been a pioneer in incorporating nutrition into the biochemistry curriculum. A graduate of the University of Rochester and the University of Oregon, Dr. Feinman has published numerous scientific and popular papers. He is the founder and former co-editor-in-chief (2004–2009) of the journal Nutrition & Metabolism. He is currently researching the application of ketogenic diets to cancer.
Comments on Cancer and Metabolism, Part 1
It's articles like this which make CrossFit feel like a kooky pyramid scheme rather the epic fitness regime that it really is! I mean I love you guys but you gotta ask why the multi billion pound cancer research industry (a good portion of which is charitable) would miss a supposedly obvious and simple alleviation of cancer. All based on the flawed keto philosophy that stone age man ate virtually no carbohydrates. This is in spite of natural carbohydrate stores in the form of root vegetables being globally accessible. Sorry this is so ranty, I work in health care and any treatment we use is so rigourously tested that these nutritionist wackos really get under my skin. Oh well, alternative therapies are just a voluntary tax on people who don't understand science.
James,
Perhaps you could quote a specific line or paragraph from Professor Feinman that you disagree with. And then, perhaps, you could explain on what logical or evidentiary basis you disagree with Professor Feinman's assertion, being sure to cite your sources. Do you think you could do that?
I ask because it seems to me you objected to an article that's not present. Thank you.
Russ,
Perhaps you could tell us your medical experience - specifically with Oncology. Also, please cite any randomized clinical trials using ketosis with/without hyperbaric oxygen that include a significant number of human patients to generate the power needed for legitimate data. The cited studies should also compare ketosis +/- HBO versus traditional cancer therapy of surgery, radiation therapy, and chemotherapy for multiple types of cancers in humans. Studies that use ketosis +/- HBO as complimentary medicine would be helpful as well.
This accumulation of meaningful data might be more helpful and also clarify for those who are not medically trained. Thank you.
Craig,
I have no medical experience and did not claim to have any. I am not an expert in oncology and did not claim to be one. If you disagree with Professor Feinman's article, great. I will direct you, though, to what I asked James above:
"Perhaps you could quote a specific line or paragraph from Professor Feinman that you disagree with. And then, perhaps, you could explain on what logical or evidentiary basis you disagree with Professor Feinman's assertion, being sure to cite your sources. Do you think you could do that?"
Russ,
I will again ask for studies in human cancer types. All of the cancer articles posted on CF.com talk about metabolic processes, discrediting accepted science, using ketosis as a cure-all to cancer (most studies quoted here have applied to glioblastomas only), and also going against NCCN Guidelines for cancer therapy. Information about NCCN Guidelines can be found here if you do not know about them - https://www.nccn.org/patients/clinical/default.aspx.
Specifically in this article, from "WHY DO WE THINK KETOGENIC DIETS WILL BE USEFUL FOR TREATING CANCER?" and on, the only examples are treatment or ketosis effects in mice models. While we can infer from mice, we are humans. What these articles promote is "If you have cancer, go for Ketosis! Forget recommended medicine!"
The article, "Use of Alternative Medicine for Cancer and Its Impact on Survival" by S Johnson et al (https://doi.org/10.1093/jnci/djx145) shows using alternative medicine as a poor choice for cancer patients in terms of survival.
So again, perhaps you could "cite any randomized clinical trials using ketosis with/without hyperbaric oxygen that include a significant number of human patients to generate the power needed for legitimate data. The cited studies should also compare ketosis +/- HBO versus traditional cancer therapy of surgery, radiation therapy, and chemotherapy for multiple types of cancers in humans. Studies that use ketosis +/- HBO as complimentary medicine would be helpful as well.
This accumulation of meaningful data might be more helpful and also clarify for those who are not medically trained. "
James, I did in vitro experiments on MCF-7 breast cancer cells and compared their metabolite profile with control MCF-12 breast cells. Giants in the field argued they weren't fermentative, by my data suggested they were (i.e. very high intracellular lactate-to-pyruvate ratio). Do you honestly think most doctors understand under redox biology well enough to even make sense of this statement? Most of the doctors I speak to, even the good ones, will admit they don't.
Just because you have a white coat, doesn't guarantee you an understanding on physiology or biochemistry.
So rather than make sweeping statements that speak to your incredulity rather than your knowledge, you may want to invest some time into actually understanding the logical and clear arguments Richard Feinman is taking the time to make.
Reply to James Lowe,
I don’t think that I was claiming that we have a simple method to alleviate cancer. While I pointed out the reasons for being optimistic and some of the research, we don’t know which, if any, cancers will be helped by ketogenic diets, which will not, or which will be made worse. Part of the problem is that we under-appreciated – all of us – the importance of ketogenic metabolism so it is important to find out the relation to cancer however it turns out. Of course, everybody has their own take on all nutritional problems. To some extent, I was trying to explain metabolism through cancer, as well as the other way around.
On the other hand, one of the major reasons for being optimistic about carbohydrate restriction for cancer is the success of the approach in treating diabetes. In many cases and depending on the extent of progression of disease, we can essentially cure the disease in an obvious if not simple way with low-carbohydrate ketogenic diets.
So, if you want to rant it might be directed at the resistance of the multi billion pound diabetes research industry (a good portion of which is charitable – get out your handkerchief) which has given itself the privilege of ignoring rigorously tested research and will, when they can, attack the researchers and clinicians personally and professionally. While the ADA is getting some approbation for their belated acceptance of low-carb diets, their “consensus” statements are unscientific, illiterate and have enough disclaimers to discourage physicians from using low-carb diets. In addition to the bad science, the effect is a good deal of patient suffering which appears to me along the lines of depraved indifference although I am not a lawyer.
I love this evidence, are you able to send me a link I can use in my assignment for bachelor of nursing so I can bring this together, I completely believe but need academic proof to put in my assignment
I love how much CrossFit is focusing on the role of nutrition in health and fitness. Thanks you! I’m a Nutritional Therapy Practitioner and this is my life!
Cancer and Metabolism, Part 1
9